Oncomedicine 2019; 4:35-42. doi:10.7150/oncm.34897 This volume Cite
Review
“Duel” and “Duet”: The Paradox of Carcinogenic Viruses and Targeted Virotherapeutics
1. Department of Chemical and Biomolecular Engineering, Vanderbilt University, Nashville, Tennessee 37235, USA. anup.p.challa@vanderbilt.edu
2. Departments of Biological Sciences, University of Memphis, Memphis, TN 38152. USA. maduco@scsk12.org
3. Departments of Pathology and Laboratory Medicine, University of Tennessee Health Science Center, Memphis, Tennessee 38163, USA. ylu@uthsc.edu
Received 2019-3-14; Accepted 2019-5-8; Published 2019-9-19
Abstract
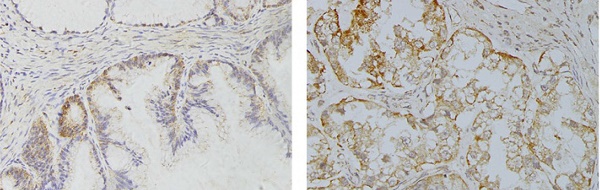
In recent years, researchers have identified seven major viruses implicated in oncogenesis, spanning across four Baltimore virological classifications. Thus, viruses are known contributors to 19 human cancers, as well as 10 chronic comorbidities known to reduce quality of life among affected patient populations. This etiology, though minor among other sources of carcinogenesis, remains an interesting area of research for oncologists and molecular biologists in its potential for understanding the complex role of exogenous oncogenes in the growth of malignant tumors.
Conversely, biomolecular researchers have seen newfound success in viral engineering: through designing natural virions to transfect tumor cells, investigators can effectively harness viral vectors for the treatment of numerous malignancies. Recent studies propose this mechanism through lytic replication within cancerous cells, allowing for destruction of neoplasms through cell death. Furthermore, adaptive and innate immune responses are stimulated in this oncolytic virotherapy, destroying virions and tumor vasculature. With responses such as these, viruses are used to battle a variety of cancers, maintaining specificity against the infection of noncancerous cells. Recent advancements also include the application of type II CRISPR-Cas9 systems in re-activation of host cell cycle controls.
Thus, the relationships between viruses and neoplasia are complex. This paper seeks to explore the implications of “duel” viruses in oncogenesis, as well as “duet” virus approaches to the treatment of disparate malignancies.
Keywords: oncogenesis, duel, duet, viruses
Introduction
The relationship between viral infection and carcinogenesis is of great modern interest; however, the ties between the two have deep historical underpinnings. Since the late eighteenth century, numerous studies [1,2,3] have revealed the possibility of transmissible etiologies among certain cancers, with the true implication of viruses attributable to Peter Rous for the identification of tumor-inducing virions [4]. Before Rous, early scientists had noted the transmissibility of certain cancers, including Italian theorist Domenico Rigoni-Stern in his phenotypic observations of increased rates of cervical cancer in married women, compared to Veronesi nuns [5,6]. Although the implicated human papilloma virus (HPV) [7] had not been discovered at this time, observations like these proved influential to the development of the field of virology.
As Rous began his viral studies in the early 1900s, he identified the retroviral pathogen now known as Rous sarcoma virus (RSV) [8] through oncological investigations in chicken. Rous observed that tumor extract filtered through sieves impermeable to wild type (WT) chicken cells and bacteria were potent in carcinogenic transfection among fowls; this was the edifice of the field of oncovirology, as Rous determined that the vector must be a “virus.” Early studies such as these, however, were often unconvincing in the scientific community for their veterinary scope of investigation. With Rous's novel framework of carcinogenic transmission, as well as the integration of epidemiological observations by earlier scientists, the discovery of the pathophysiology of human viral infection brought oncoviruses to the forefront of molecular biology research [9]. With scientists like Dulbecco [10], Temin [11], and Baltimore [12], biologists discovered the mechanism of retroviral transfection of human cells; furthermore, the novel identification of viral oncogenes in 1989 led to the Nobel Prize for researchers J. Michael Bishop [13,14] and Harold E. Varmus [15].
Hence, modern virology expanded its reach into cancer biology. Viruses are known carcinogens, mostly for their role in negotiating oncogene transfer through infection of healthy cells [16]. Lysogenic virions can thus embed oncogenic DNA into healthy cellular sequences, resulting in tumorigenesis through general dysregulation of the cell cycle. Lytic viruses may also promote carcinogenesis, through which transfection results in the translation of viral oncoproteins (PI3K, e.g.); this can result in unchecked cell growth and subsequent malignancy, as tumorigenesis and repeat infection are simultaneously promoted [17].
Alternatively, oncolytic virotherapy (OV) is a new avenue in the treatment of numerous cancers [18]. This approach involves the injection of a lytic virus with high specificity for cancerous cells; thus, the virus can reduce malignancy through lysis, as tumor cells are destroyed though the production of daughter virions. As viral replication and cell lysis occur, immune pathways are triggered to both destroy the virus and attack the tumor in which the virus breeds. Hence, OV allows for the destruction of tumors through simultaneous immunological attack of the transfecting virus. This novel approach also has historical underpinnings: as early as the 1950s, oncologists noticed an improvement in the general health of their patients upon vaccination, presumably from a similar, more muted phenomenon. This effect was also noted upon infection from viruses not empirically implicated in cancerous symptoms [19].
Hence, the bi-faceted role of viruses in modern molecular biology involves dissection of their infectious and palliative properties. With the development of OV, researchers have harnessed both genetically-engineered vectors and virions naturally attracted to malignant neoplasms (i.e., viruses non-pathogenic in healthy tissues). Yet, viral etiologies account for almost 15% of malignancies worldwide, with significantly increased incidence in areas where implicated virions are attributable to other epidemic diseases [17].
“Duel”: Carcinogenic Viruses and Associated Diseases
According to recent estimates, one in six cancers is attributable to a viral etiology; in developing nations, where density of infection by implicated virions is increased, the carcinogenic factor reaches nearly 50% [17]. Malignancies are mapped to seven major viruses, spanning across four Baltimore viral groups [17,20]. Hence, the implicated viruses include Epstein-Barr virus (EBV) [21], hepatitis B virus (HBV) [22], human T-lymphotropic virus (HTLV-1) [23], HPV [24], hepatitis C virus (HCV) [25], Kaposi sarcoma herpesvirus (KSHV) [26], and Merkel cell polyomavirus (MCV) [27], as summarized in Table 1 and Table 2.
Taxonomy and Baltimore classifications of seven virions implicated in carcinogenesis
Virus | Family | Baltimore Classification |
---|---|---|
EBV | Herpesviridae | dsDNA |
HBV | Hepadnaviridae | dsDNA-RT |
HTLV-1 | Retroviridae | ssRNA-RT (positive strand) |
HPV | Papillomaviridae | dsDNA |
HCV | Flaviviridae | ssRNA-RT (positive strand) |
KSHV/HHV8 | Herpesviridae | dsDNA |
MCV | Polyomaviridae | dsDNA |
Adapted from reference [17]
Implicated viruses and their associated neoplasia and comorbidities
Virus | Neoplasms | Comorbidities |
---|---|---|
EBV | 1. Burkitt's lymphoma 2. Diffuse large B-cell lymphoma 3. Hodgkin lymphoma 4. Undifferentiated nasopharyngeal carcinoma 5. Gastric adenocarcinoma 6. Leiomyosarcoma 7. Post-transplant lymphoproliferative disorder (PTLD) | 1. Infectious mononucleosis 2. X-linked lymphoproliferative syndrome (Duncan's syndrome) |
HBV | Hepatocellular carcinoma | 1. Hepatitis 2. Cirrhosis |
HTLV-1 | Adult T-cell leukemia | HTLV-1 myelopathy/tropical spastic paraparesis (HAM/TSP) |
HPV | 1. Cervical carcinoma 2. Squamous cell head and neck cancer 3. Squamous cell anal cancer 4. Vulvar cancer | N/A |
HCV | 1. Hepatocellular carcinoma 2. Rare lymphomas (suspected) | 1. Hepatitis 2. Cirrhosis |
KSHV/HHV8 | 1. Kaposi's sarcoma 2. Primary effusion lymphoma 3. Multicentric Castleman disorder | N/A |
MCV | Merkel cell carcinoma | N/A |
Adapted from reference [17]
For these viruses, the pathophysiology of infection involves unique mechanisms of transfection and viral integration. However, on the whole, oncoviruses are similar in the progression of their infection, involving injection of vDNA, episomal integration of the viral genome into that of the host, and transcription and subsequent translation of vDNA in the promotion of tumorigenesis. Consider the following example of EBV-associated oncogenesis:
EBV: Epstein-Barr virus is highly transmissible, contracted through contact with infected saliva, semen, or vaginal fluid of an affected individual [28]. Viremia is associated with infection of epithelial cells, as well as B-lymphocytes; furthermore, infection presents differentially on the basis of cell type. In epithelial cells, EBV exhibits both lysis and lysogeny, with lysogenic preference. Thus, as EBV undergoes lytic replication in nasopharyngeal cells, virion number is significantly increased, facilitating further infection of B-lymphocytes. Nonetheless, as lysogeny occurs in epithelial tissues, expression of viral oncogenes (vDNA) allows for neoplasia through upregulation of proliferative signaling pathways and simultaneous downregulation of apoptosis. Infection of B-lymphocytes shows high preference for lysogeny, in which the viral genome is integrated into healthy, immune sequences; in these cells, as well, proliferation is promoted through expression of vDNA. Specifically, EBV lysogeny occurs through episomal pathways: vDNA within the viral sequence is easily transcribed without transcription of the cell's original genome, allowing for oncogenic interference within proliferative and apoptotic signaling pathways. Differential genetic expression is also implicated in these pathways; thus, scientists have identified four variants of latency implicated in EBV lysogeny. Differential expression of the viral genes EBER, EBNA1, LMP1, LMP2A, EBNA2, and EBNA3 allow for presentations of disparate malignancies. These genes confer upon viral tumors resistance to proliferative downregulation by the host's own machinery, in addition to promoting cell division. With this, researchers have categorized latency on the basis of genetic expression. Latency scheme 0 sees expression of the EBER and LMP2A gene families, which is associated with development of B-cell malignancies. Latency scheme I, associated with Burkitt's lymphoma, sees expression of the EBER and EBNA1 genes only, with expression of EBER, EBNA1, LMP1, and LMP2A genes involved in latency II and the development of Hodgkin's lymphoma. Latency III is associated with expression of all aforementioned vDNA and the subsequent development of PTLD. Latency scheme IV is largely investigational, with expression of several viral genes still undetermined. Nonetheless, this latency scheme is associated with the development of PTLD and an infectious comorbidity of mononucleosis. Upon immunosuppression-either through contraction of another comorbidity or treatment with agents like methotrexate-EBV triggers lysis, disrupting all latency schemes [29].
Though viruses maintain high specificity in their mechanisms of infection, most of the above virions follow generalized schemes of carcinogenic transfection analogous to that described in EBV. Most important in this transfection process is the promotion of cell division, with simultaneous suppression of apoptotic pathways. This involves both gain-of-function (GOF) in the promotion of tumorigenesis, as well as loss-of-function (LOF) through the sequestration of host cell proteins in virally-mediated disruption of the cell cycle [22,25,26,27,30,31]. Starting with RSV, researchers have thus identified a myriad of oncogenes-including those listed above-capable of episomally triggering oncogenesis [4,29]. Although concentration of virion molecules implicated in carcinogenic viremia is dependent upon viral identity, this pathway of disequilibrium is the dominant factor of oncoviruses.
“Duet”: Oncolytic Virotherapy
Paradoxically, viruses are implicated in both the treatment of malignant neoplasia and its genesis [32]. Dissecting the immunotherapeutic value of virions first requires a discussion of virology in the age of high-throughput biology.
Viral engineering is characterized by its specificity in targeting tumor cells; furthermore, engineered systems have high sensitivity for specific neoplasms, by which bioengineers manipulate cell signaling affinities in the selection of adaptable viral characteristics [33]. This first requires high-precision diagnostics. Clinically, bioinformatic advancements have promised the development of viral diagnostics more accessible to “benchtop” re-engineering. With the rise of whole exome sequencing, conventional biopsy methods have been adapted through the introduction of sequencing and amplification, as well as database-aligned analysis for pinpointing etiology more accurately [34]. Under this scheme, initial methods of tumor pathology remain the same: extracted tissue is first flash frozen after biopsy, followed by microscopic definition of tumor boundaries. Next, nucleic acids from tissue high in tumor content is extracted and purified; the genetic material (DNA, mRNA, total RNA) is then amplified with PCR and sequenced. The choice of sequencing specificity involves researcher interest: in DNA sequencing, the researcher is unable to analyze retroviral infection; however, transcriptomic sequencing ignores DNA lysogeny. Total RNA sequencing encompasses both retroviral infection and transcriptomic viral elements; however, it continues to ignore DNA-mediated latency. Thus, with whole genome sequencing (WGS), researchers have found holistic success in approaching viral genetics [35].
Using sequencing libraries, bioinformaticians then analyze WGS reads and separate them into human and non-human components. The determination of non-human sequences in the tumor sample implies a viral etiology of transfection. With this information, software like Basic Local Alignment Search Tool (BLAST) [36] then allow for the comparison of viral reads to existing sequence data, facilitating a diagnosis and understanding of oncological pathophysiology. Furthermore, this approach allows for the recognition of new infectious particles through analysis of genetic deviance from known viral sequences; this “evolutionary” approach to sequence processing thus allows for an understanding of viral mutation and its relationship to carcinogenesis, as viruses continue to present one of the highest mutation rates among microbes [35].
Armed with information on specific viral sequences, the translational cycle [37] can spin towards the bioengineering “benchtop,” on which researchers are able to prepare OV. The preparation of this “cancer biowarfare” involves two (2) specific signaling mechanisms: preferential infection for cancerous cells-with no infection in WT host cells-as well as activation of host immune responses against the malignant neoplasm upon tumor cell lysis. Successful specificity in viral transfection relies upon toll-like receptor (TLR) pathways and interferon signaling: in virally-infected cancer cells, TLR and interferon signaling pathways are disrupted, allowing oncolytic virotherapy to function in infecting the tumor. Contrastingly, healthy host cells observe no disruption in immunological signaling, preventing OV-mediated infection. Upon infection, the oncolytic virus allows for tumor lysis, triggering host immune response through the detection of neoantigens from the malignancy. Pattern-associated molecular patterns (PAMPs), including vDNA, as well as damage-associated molecular patterns (DAMPs) from cell lysis, trigger the IL-2 signaling pathway, resulting in the dispatch of CD4+ and CD8+ cytotoxic T cells, effectively attacking the cancer. Further release of alert cytokines, including TNF-𝛼 and other interleukin molecules, results in stimulation of innate immune responses; with subsequent downregulation of MHC-1 production, further activation of innate and adaptive host immunity results in effective destruction of malignant cells and viral material [38].
Current OV clinical trials have revealed success in virotherapeutics, including both intra-arterial and intratumoral administration of the therapy [39]. Currently, most trials remain phase I and phase II studies of commercialized virions. In a novel phase III trial of T-vec, a re-engineered HSV-1 with mutations in the 𝛾34.5 and 𝛼47 genes and insertion of human granulocyte-macrophage colony-stimulating factor (GM-CSF) at the 𝛾34.5 locus, researchers observed no fatal treatment-related adversities associated with T-vec treatment in a cohort of melanoma patients. This trial was the first phase III study to demonstrate that intralesional OV therapy was successful in limiting metastasis and tumor growth, as well as increasing observed survivorship through stimulation of host immune responses.
With this noted success, researchers have since developed numerous other OV approaches, including genetically-engineered adenovirus (CG0070) and triple-mutated HSV-1. Drawing on the success of T-Vec, researchers developed G47∆, including an insertion of the E. coli lacZ gene in the viral genome; this added third mutation has seen much success in treating glioblastoma, among other tumors of the central and peripheral nervous systems, earning it the title Sakigake (“ahead of the world”) by the Ministry of Health, Labour and Welfare, Japan. OV advancements are cumulative, and the widespread success of lacZ insertion in re-engineering herpes virus has since led to heightened development of similar vectors.
Also of recent interest is the development of naturally oncolytic viruses. Biologists encountered difficulty in finding WT viruses suitable for OV during initial searches in the 1960s; however, with the “engineering mindset” developed through the genesis of synthetic OVs, this pursuit has gained new momentum. Thus, researchers have recently harnessed reoviruses for their therapeutic potential: these virions are good OV candidates, as they multiply solely through transfection of transformed cells. This suggests that host viremia is highly unlikely, while the oncolytic potential of the drug is exploited. With this, manufactured reovirus (Reolysin) has been approved by the Food and Drug Administration (FDA) for treatment of malignant glioma, ovarian cancer, and pancreatic cancer.
A summary of current OV clinical trials is presented in Table 3 [39]. These approaches are inherently passive, involving the administration of exogenous biomolecules in an attempt to combat neoplasia [40]. In contrast, active immunotherapeutic approaches in cancer vaccination, by which immunostimulatory agents are administered to upregulate immune response in an affected patient, thereby increasing the likelihood of attack on neoplasia [41]. Cancer vaccines are made specific in the choice of injected antigen; for instance, in pancreatic cancer, patients are immunized with telomerase upon detection of infection. Because telomerase is often ejected by pancreatic tumors, treatment with this protein “primes” the immune system for attack of pancreatic neoplasms. Other common vaccine targets include the injection of tumor itself, by which immunity is not solely upregulated: in colon cancer, for instance, increased antibody titers post-treatment with tumor cells promotes an enhanced disease-fighting response, while the choice of colorectal cells for injection underlies the specificity of observed cytotoxic responses. Adjuvants to these therapeutics are also developed and delivered with their corresponding active therapies, as discussed above [42]. Thus, as OV approaches are optimized for efficiency, active cancer immunotherapies continue to grow tangentially as viable oncotargets for drug manufacturing.
Challenges to OV
As above, the theory behind passive immunotherapeutics presents a viable, ever-more popular option in cancer treatment that will soon be readily available for cancer patients as a treatment option. However, a significant hurdle to OV involves diminishment of therapeutic potential through reducing antibody-mediated neutralization of administered viruses. While genetically-engineered virions may be designed against neutralization, natural viremic particles, including the highly-effective reovirus, are highly susceptible to attack by circulating B-cells. This nearly invalidates the use of natural virions in OV, owing to high rates of recurrence and near-complete tumor regrowth observed in some trials within three (3) weeks of reovirus administration.
Other problems with OV involve differentiation in immune response, dependent on site of injection. Some studies have documented difference in tumor regeneration upon the injection of reovirus within intra-arterial and intralesional positions [39]. This difference must be further evaluated in the development of administration strategies and drug optimization, as OV continues to occupy a position in the forefront of modern oncology.
Future of OV and Its Limitations
The above-described innovations in passive and active cancer immunotherapy have been generalized for all neoplasia; however, recent innovations have probed the development of therapeutics specific for the development of virally-driven cancers. In turn, the specificity of Clustered Regularly Interspaced Short Palindromic Repeats (CRISPR)/Cas9 probes lends itself to the development of more generalized therapies for oncogene-addicted tumor models.
Carcinogenic viruses are inherently oncogene-addicted [43], by which tumorigenesis stems from expression of one or more episomes towards dysregulation of the host cell cycle. Because viral oncogenes are mostly well-characterized and transform healthy cells through an episomal method of inheritance, they are prime candidates for new gene-editing approaches to immunotherapeutics, which seek to prevent oncogene addiction from its onset by disrupting the process of episomal inheritance.
Current prominent trials in oncolytic virotherapy
Therapeutic | Virus | Modified Gene(s) | Inserted Gene(s) | Disease | Approval Status |
---|---|---|---|---|---|
T-Vec (imlygic, talimogene, laherparepvec) | HSV-1 | 1. 𝛾34.5 2. 𝛼47 | GM-CSF | Unresected melanoma, stage IIIB-IV | *Approved in United States in 2015 *Approved in Europe in 2016 |
G47∆ | HSV-1 | 1. 𝛾34.5 2. 𝛼47 3. ICP6 | lacZ | Glioblastoma | *Phase II trial initiated in 2015 *Named Sakigake |
JX-594 (pexa-vec, pexastimogene, devacirepvec) | Vaccinia virus | HSV-TK | • GM-CSF • lacZ | Advanced stage hepatocellular carcinoma | *Phase III trial started in 2015 |
CG0070 | Adenovirus | 1. E1A 2. E2F-1 promoter | GM-CSF | Non-muscle invasive bladder cancer [after failure of Bacillus Calmette-Guerin therapy (BCT)] | *Phase II/III trial ongoing in patients with bladder cancer |
Reolysin (pelareorep) | Reovirus | N/A | N/A | Metastatic and/or recurrent head and neck cancer | *Phase III trials completed *Granted orphan drug designation by United States Food and Drug Administration (FDA) |
Adapted from reference [39]
Herein lies the “duel” and “duet” paradox of viruses in cancer: while carcinogenic viruses may promote neoplasia, virions also have application in precision cancer therapeutics. A prime example of the latter is the development of the Type II RNA-mediated CRISPR/Cas9 gene editing system [44] as a viable cancer treatment. This endonuclease-mediated editing scheme involves the application of prokaryotic immunity to the induction of both LOF and GOF mutations within growing tumors, upregulating the activity of necrotic factors and oncogenes, while further disabling the transformational power of oncogenes and viability of existing tumors. The natural Type II CRISPR/Cas9 system functions through insertion of exogenous, transfected DNA into the host genome; these protospacers‒located near specific palindromic genetic repeats‒are then transcribed into crRNA, along with ambient host DNA. This molecule, along with a Cas9-type endonuclease and tracrRNA molecule, is clustered to form the CRISPR/Cas9 gene editing system. This CRISPR/Cas9 complex is then readily available for use in gene editing, and phage delivery vehicles can deliver the CRISPR complex to a eukaryotic cell. Subsequently, the crRNA readily binds to a complementary host DNA read (i.e., the sequence of the protospacers), locking the CRISPR/Cas9 complex to the host genome. Cas9 endonuclease is then able to induce a double-stranded break (DSB) in the host DNA; the repair of this cut is then facilitated in two (2) ways: through non-homologous end joining (NHEJ) or homology-directed repair (HDR). In the former, the host attempts to rectify the break with DNA polymerase II systems; however, the repair is often imperfect and is highly subject to indel mutations, rendering potential LOF of a specific host function through frameshift. Conversely, HDR involves the introduction of a new sequence (allowing for the formation of a guide RNA (gRNA) complex) to seal the DSB. This renders new functionality on the host, allowing GOF.
This CRISPR/Cas9 targeting approach involves genetic editing of transformed cells, through which phage-delivered editing machinery is harnessed in disrupting oncogene addiction [43]. An example of this technique is given for cervical cancer, which is often incident upon infection with HPV:
Molecular probes have identified the expression of oncoproteins E6 and E7 as crucial regulators of transformed cell survival in cervical neoplasia; repression of these proteins has been implicated in apoptosis. When E6 is inactivated, expression of the p53 tumor suppressor gene is re-facilitated, allowing for regain of cell-cycle control; similarly, inactivation of E7 is implicated in re-activation of the retinoblastoma protein (pRb) pathway, allowing for subsequent senescence. The exploitation of this pathway before the introduction of CRISPR-Cas9 genome editing called for the application of various non-biological techniques, including the application of antisense knockdown through generation of phosphorothioate oligonucleotides in the repression of E6/E7 translation, as well as biological intervention, such as the injection of WT p53 into transformed cell lines. Further techniques included treatment of transformed cell lines with zinc-ejecting agents, thus disrupting the crucial binding of E6 and E7 translations to E6-associated protein (E6AP) and E7-associated protein (E7). This association is a key step in the inactivation of p53 signaling; thus, omission of zinc and subsequent charge incompatibility in protein binding results in continued functionality of p53 pathway elements, leading to apoptosis. With the rise of new biotechnology techniques, RNA interference (RNAi) has allowed for the engineering of short-interfering RNAs (siRNA) and short-hairpin RNAs (shRNA) in the silencing of E6/E7 gene expression. CRISPR/Cas9 now provides a more modern alternative to previous biochemical injection, in which NHEJ is used to silence E6/E7 gene expression through injection of WT Cas9 in antisense knockout. Given the relative shortness of E6/E7 genes, other editing approaches involve regulation of transcriptional and translational elements, specifically at the post-transcriptional level. Furthermore, an intuitive approach involves activation of systemic immunity, through which a transcript is injected with the CRISPR/Cas9 machinery to promote expression of RNA-stabilizers important to the generation of viral capsid proteins [45]. The subsequent reassembly of virions thus triggers host immunity, leading to loss of pathogenicity [43].
CRISPR-based treatments, however, remain limited in their implementability. Major limitations center around target specificity, such as off-target binding of Cas9 endonuclease, as well as activation of host immunity against delivery vectors. Deficiencies in target binding are largely attributable to recent observations of gRNA in genome editing: gRNA-mediated editing is feasible without complete complementary base pairing between gRNA and the host sense strand. Nonetheless, by reducing the size of the guide complex to 20 nt or less, specificity is largely restored; other approaches have included the introduction of point mutations within the Cas9 active site, allowing for reduced binding to the phosphate backbone to reduce promiscuity. Similarly, mutations can be induced to allow for editing only upon binding of an additional enzyme to the host DNA; this requirement allows Cas9 activation only upon complexing with an enzyme with enhanced binding specificity, thus regulating the introduced DSB in the host genome. While these techniques have shown thousandfold increase in editing specificity, they have also been implicated in reductions in efficiency, whereby the additional requirements against promiscuity have resulted in diminished endonuclease activity by the Cas9 protein.
With these issues, CRISPR therapeutics may result in further amplification of neoplasia, in which combination oncotherapeutics may be less effective; this is attributable to undesired DSBs, which may induce resistance to previously-prescribed treatments on the genetic level. Thus, CRISPR-guided oncotherapy must be accompanied with routine patient sequencing in order to monitor the presence of deleterious indels; this, in itself, presents an element of complication, making responsible CRISPR therapeutics inaccessible to patients without access to sequencing technologies [43].
Conclusions and Recommendations
There is exciting work underway in the mechanistic detail of virally-driven oncogenesis and broad-spectrum virotherapy. Given the multifactorial nature of most cancers, combination therapies, targeting multiple stages of cell cycle dysregulation, metastasis, and host immune response, provide a holistic approach to treatment, in which virotherapeutics are soon likely to make a major appearance. This is evident in the progression of recent clinical trials, in which techniques like CRISPR/Cas9 have now been tested in small cohorts of human subjects: in an ongoing trial at the University of Pennsylvania, researchers have developed the first human study investigating CRISPR-mediated T-cell immunostimulation in the clearance of multiple myeloma [46,47].
Thus, the development of virally-inspired oncotherapeutics is a promising area of advancement in modern bioengineering. With the rise of personalized medicine, techniques like HDR and NHEJ CRISPR/Cas9, which are largely driven by infectious genomics and patient sequences themselves, are ever-more relevant and likely to expand in the coming years. For this transition to be successful, however, more research must be documented across both the “bench” and “bedside”: more clinical trials showing promising results on existing virotherapies should be expanded towards Phase III, and problems with specificity and efficiency of tools like CRISPR/Cas9 must be resolved before they can become more feasible trial candidates.
With the development of more virally-driven therapeutics and the further elucidation of mechanisms of carcinogenic transfection, the future looks bright for the continuing integration of virology in the study and treatment of malignant neoplasm.
Competing Interests
The authors have declared that no competing interest exists.
References
1. Sudhakar A. History of cancer, ancient and modern treatment methods. J Cancer Sci Ther. 2009;1:1-4
2. Blackadar CB. Historical review of the causes of cancer. World J Clin Oncol. 2016;7:54-86
3. Plimmer HG. The parasitic theory of cancer. Br Med J. 1903;2:1511-1515
4. Rubin H. The early history of tumor virology: Rous, RIF, and RAV. Proc Natl Acad Sci USA. 2011;108:14389-14396
5. DiMaio D. Nuns, warts, viruses, and cancer. Yale J Biol Med. 2015;88:127-129
6. Griffiths M. “Nuns, virgins, and spinsters”. Rigoni-Stern and cervical cancer revisited. Br J Obstet Gynaecol. 1991;98:797-802
7. Paavonen J. Human papillomavirus infection and the development of cervical cancer and related genital neoplasias. Int J Infect Dis. 2007;11(Suppl 2):S3-S9
8. Lounková A. et al. Molecular events accompanying Rous sarcoma virus rescue from rodent cells and the role of viral gene complementation. J Virol. 2014;88:3505-3515
9. Weiss RA, Vogt PK. 100 years of Rous sarcoma virus. J Exp Med. 2011;208:2351-2355
10. Verma I. Renato Dulbecco (1914-2012). Nature. 2012;483:408
11. Temin H, Mizutani S. Viral RNA-dependent DNA polymerase: RNA-dependent DNA polymerase in virions of Rous sarcoma virus. Nature. 1970;226:1211-1213
12. Friedberg E. An interview with David Baltimore. Nat Rev Mol Cell Biol. 2008;9:670-671
13. Payne GS, Bishop JM, Varmus HE. Multiple arrangements of viral DNA and an activated host oncogene in bursal lymphomas. Nature. 1982;295:209-214
14. Eilers M. et al. Chimaeras of Myc oncoprotein and steroid receptors cause hormone-dependent transformation of cells. Nature. 1989;240:66-68
15. The 1989 Nobel Prize in Physiology or Medicine is awarded to J. Michael Bishop and Harold E. Varmus for their contribution to cancer research. Essay Inf Sci. 1990;13:3-10
16. Weinberg RA. Interview: Oncogenes. CA: Can J Clin. 1983
17. Chang Y, Moore PS, Weiss RA. Human oncogenic viruses: nature and discovery. Phil Trans R Soc B. 2017:324
18. Fountzilas C, Patel S, Mahalingam D. Review: Oncolytic virotherapy, updates and future directions. Oncotarget. 2017;8:102617-102639
19. Chiocca EA, Rabkin SD. Oncolytic viruses and their application to cancer immunotherapy. Cancer Immunol Res. 2014;2:295-300
20. Sigalov A. The school of nature IV. Learning from viruses. Self/Nonself. 2010;1:282-298
21. Fagundes Gequelin LC. et al. Epstein-Barr virus: General factors, virus-related diseases and measurement of viral load after transplant. Rev Bras Hematol Hemoter. 2011;33:383-388
22. Liang TJ. Hepatitis B: The virus and disease. Hepatology. 2009 (Suppl 5): S13-S21
23. Gonçalves DU. et al. Epidemiology, treatment, and prevention of Human T-cell leukemia virus type 1-associated diseases. Clin Microbiol Rev. 2010;23:577-589
24. Braaten K, Laufer M. Human Papillomavirus (HPV), HPV-related disease, and the HPV vaccine. Rev Obstet Gynecol. 2008;1:2-10
25. Li HC, Lo SY. Hepatitis C virus: virology, diagnosis and treatment. World J Hepatol. 2015;7:1377-1389
26. Mariggio G, Koch S, Schulz TF. Kaposi sarcoma herpesvirus pathogenesis. Phil Trans R Soc B. 2017:372
27. Liu W, MacDonald M, You J. Merkel cell polyomavirus infection and Merkel cell carcinoma. 2016; 20: 20-27.
28. Cohen JI. Epstein-Barr virus infection. N Engl J Med. 2000;343:481-492
29. Ali AS. et al. Epstein-Barr virus: Clinical and epidemiological revisits and genetic basis of oncogenesis. Open Virol J. 2015;9:7-28
30. Ishiji T. Molecular mechanism of carcinogenesis by Human Papillomavirus-16. J Dermatol. 2015;27:73-86
31. Boxus M, Willems L. Mechanisms of HTLV-1 persistence and transformation. Br J Cancer. 2009;101:1497-1501
32. Russell SJ, Peng K, Bell JC. Oncolytic virotherapy. Nat Biotechnol. 2012;30:658-670
33. Kelley E, Russell SJ. History of oncolytic viruses: Genesis to genetic engineering. Mol Ther. 2007;15:651-659
34. Kellam P. Post-genomic virology: The impact of bioinformatics, microarrays, and proteomics on investigating host and pathogen interactions. Rev Med Virol. 2001;11:313-329
35. Tang K, Larsson E. Tumor virology in the era of high-throughput genomics. Phil Trans R Soc B. 2017:372
36. National Center for Biotechnology Information: Bethesda, MD, United States of America. BLAST: Basic Local Alignment Search Tool. Updated 18 May 2018. https://blast.ncbi.nlm.nih.gov/Blast.cgi
37. Ledford H. The full cycle. Nature. 2008;453:843-845
38. Newman JH, Zloza A. Infection: A cause of and cure for cancer. Curr Pharmacol Rep. 2017;3:315-320
39. Fukuhara H, Ino Y, Todo T. Oncolytic virus therapy: A new era of cancer treatment at dawn. Cancer Sci. 2016;107:1373-1379
40. Walker LM, Burton DR. Passive immunotherapy of viral infections: “Super-antibodies” enter the fray. Nat Rev Immunol. 2018;18:297-308
41. Chodon T, Koya R, Odunsi K. Active immunotherapy of cancer. J Immunol Invest. 2015;8:817-836
42. Melero I. et al. Therapeutic vaccines for cancer: An overview of clinical trials. Nat Rev Clin Oncol. 2014;11:509-524
43. Jubair L, McMillan NAJ. The therapeutic potential of CRISPR/Cas9 systems in oncogene-addicted cancer types: virally driven cancers as a model system. Mol Ther Nucleic Acids. 2017;8:56-63
44. Jinek et al. A programmable dual-RNA-guided DNA endonuclease in adaptive bacterial immunity. Science. 2012;337:816-821
45. Faridi et al. Oncogenic potential of Human Papillomavirus (HPV) and its relation with cervical cancer. Virol J. 2011;8:269
46. Kaiser J. http://www.sciencemag.org/news/2016/06/first-proposed-human-test-crispr-passes-initial-safety-review
47. U.S. National Library of Medicine: Bethesda, MD, United States of America. NY-ESO-1-redirected CRISPR (TCRendo and PD1) Edited T Cells (NYCE T Cells). Revised 06 June 2018. https://clinicaltrials.gov/ct2/show/NCT03399448
Author contact
Corresponding author: Yi Lu, Ph.D., Department of Pathology and Laboratory Medicine, University of Tennessee Health Science Center, Cancer Research Building, 19 South Manassas Street, Memphis, TN 38163, USA. E-mail: yluedu